3 Rock-Solid Predictions (j/k) About What To Expect From Photonics In 2024

By John Oncea, Editor
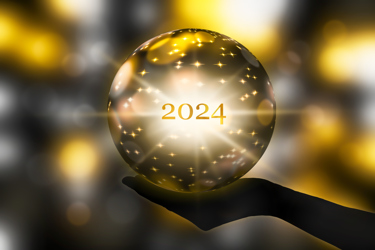
Before 2023 comes to an end we want to go on record with three things that we guarantee will happen in 2024.
(Guarantee not valid anywhere, anytime, and/or any place. Void everywhere.)
Looking back is easy. Predicting the future? In the words of Donnie Brasco, “Fuhgeddaboudit (NSFW).”
But, I’ve been tasked with looking into the ol’ crystal ball and putting my ass in the jackpot (also NSFW) by telling you what 2024 is going to bring to the industry. So, sit back and enjoy what follows, one editor’s best guesses at what direction photonics – a rapidly evolving and constantly changing industry, by the way – is poised to take. *
* For entertainment purposes only. No wagering.
Photonics are crucial in data transmission and will play a pivotal role in enabling quicker data transfer rates and reducing latency in networks.
“With the growth in artificial intelligence (AI), 5G systems, cloud computation, and the Internet of Things, transmitters with extremely high capacities are required for data communication,” writes Phys.org. Ultrafast optical modulation is crucial for high-capacity transmitters and significant progress has been made using various material systems and mechanisms to develop high-speed optical modulators.
Now comes word that a team of scientists from Zhejiang University have developed a compact lithium-niobate-on-insulator (LNOI) photonic chip. “Optical modulators on an LNOI platform have exhibited great potential,” Phys.org writes. “This arises from a linear electro-optic (EO) effect, low excess loss, and high stability.”
LNOI modulators that employ Mach-Zehnder interferometers (MZIs) and microresonators have demonstrated excellent performance. The length of the phase-shifting arms is usually several millimeters or even centimeters to achieve a low voltage. To enable high-speed operation, traveling-wave electrodes have been introduced along with high-speed MZIs. However, the large size of the arraying LNOI MZIs makes them inconvenient for high-capacity multiplexed systems with multiple channels.
“Alternatively, the resonator-based modulators of LNOI can be compact, which may reduce power consumption,” notes Phys.org. “Therefore, resonator-based LNOI modulators have attracted significant attention recently.”
The research team has successfully demonstrated high-speed LNOI modulators based on a specific 2 × 2 Fabry-Perot (FP) cavity. This enables an ultra-high 3-dB bandwidth beyond 110 GHz and data capacity of up to 140 Gbps. The size of the modulation section is much smaller than that of the reported LNOI ring resonator modulators, which makes it very attractive for arraying wavelength-division multiplexing (WDM) systems.
Advanced multiplexing techniques have been extensively researched to increase link capacity, by sending data through multiple channels concurrently. One such successful technique is Wavelength Division Multiplexing (WDM), which employs multiple wavelength channels. Over the years, different waveguide structures have been created to design WDM filters with superior performance.
Advancements in photonics will lead to more stable and scalable quantum computing systems, revolutionizing computation as we know it.
Photonics, with its ability to control and manipulate light at the quantum level, is a promising avenue for the development of quantum computing technologies. Its unique properties enable researchers to explore and implement various aspects critical for the advancement of quantum computation.
“Photonic quantum computing is an approach that relies on the use of photons as quantum bits, or qubits,” writes diginomica. “Photons are particles of light and can be manipulated and controlled to carry and process quantum information. In photonic quantum computing, qubits are encoded in the quantum states of photons.”
There are two commonly used approaches for encoding qubits in photons- polarization and path encoding. In polarization encoding, the quantum states of photons are determined by the orientation of their electric field oscillations. For instance, horizontal polarization represents |0〉 and vertical polarization represents |1〉. On the other hand, in path encoding, qubits are encoded based on the spatial paths that photons take. For example, taking one path represents |0〉 while taking another path represents |1〉.
Photons possess various advantages for quantum computing. Firstly, they can travel long distances without significant loss of information, which is crucial for constructing large-scale quantum networks. Secondly, photons are relatively immune to environmental noise and can maintain their quantum states over more extended periods compared to other physical systems. Moreover, they can be conveniently manipulated using linear optical components, such as beam splitters and phase shifters.
“One of the main differences between photonic qubits and physical qubits, such as those based on superconducting circuits or trapped ions, is the way they are manipulated and measured,” diginomica writes. “In photonic quantum computing, operations on qubits are typically implemented using linear optical components, and measurements are performed using photodetectors. This contrasts with physical qubits, where operations are achieved through the manipulation of the physical properties of the system, such as the energy levels of superconducting circuits or the internal states of ions.”
There are different ways in which qubits are connected, depending on the physical system used. In physical qubit systems, qubits can interact directly with each other due to their physical properties, which allows the implementation of two-qubit gates. However, in photonic systems, qubits do not naturally interact with each other. Instead, quantum gates are implemented by manipulating the states of individual photons, and additional resources such as entangled photon pairs or quantum memories are used to enable interactions between distant qubits.
The current buzz around photonic technology is that photon qubits can operate at room temperature, eliminating the need for cooling. However, in most practical applications of photonic quantum computers, the photons themselves are not cooled to very low temperatures. The waveguides, beam splitters, and detectors used to manipulate and detect photons, on the other hand, frequently use materials that require cryogenic temperatures. Photodetectors and other components in photonic quantum computing are typically made from superconducting materials that operate at temperatures close to absolute zero (about 0.01 Kelvin). Cooling the components to these extremely low temperatures helps to minimize thermal noise and improve the coherence and stability of the manipulated quantum states.
“So, while photons themselves may not require extreme cooling, the supporting infrastructure and components in photonic quantum computers still need to be cooled to cryogenic temperatures to achieve the necessary quantum coherence and maintain the fragile quantum states required for quantum computation,” writes diginomica.
The application of biophotonics will lead to breakthroughs in understanding biological systems, aiding in drug development, neuroimaging, and personalized medicine.
Biophotonics is a multidisciplinary research area that uses light-based technologies for medicine and life sciences and has the potential to advance medical diagnostics, therapeutics, and biotechnology. The future of biophotonics will likely continue to transform various aspects of biology and healthcare by enabling more precise, non-invasive, and comprehensive ways to study, diagnose, and treat diseases while offering deeper insights into the complexities of living systems.
Biophotonics is currently pushing the limits of resolution in imaging techniques like fluorescence microscopy, enabling visualization of cellular structures and processes in unprecedented detail. It is also playing a role in the development of high-speed imaging methods that capture biological processes in real time at the cellular and molecular levels, allowing for dynamic observations.
Photodynamic therapy (PDT) and light-based treatments are being rolled out, allowing for more targeted and precise treatment of diseases including cancers and microbial infections. This has the potential to deliver more effective treatments for neurological disorders and understanding of brain function.
The development of portable and low-cost biophotonic devices for rapid and accurate diagnosis of diseases, enabling point-of-care testing in various settings, will improve diagnostics. In addition, biophotonics will enhance the sensitivity and specificity of sensors for detecting biomarkers, pathogens, and other biological entities with high precision and speed.
Advancements in miniaturization and the integration of biophotonic components onto small chips will result in lab-on-chip platforms that will enable complex biological assays and analyses in compact and automated systems. In addition, wearable tech will gain in popularity thanks to the integration of biophotonic sensors and devices into wearable technologies for continuous monitoring of health parameters and early disease detection.
Finally, quantum biophotonics will boom, leveraging quantum properties of light for ultrasensitive detection of biological molecules and cellular processes, opening new avenues for diagnostics and research.