LED Light Source: Major Advance In Fluorescence Microscopy
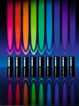
A Radically New Approach
Fluorescence microscopy requires an intense light source at the specific wavelength that will excite fluorescent dyes and proteins. The traditional method employs a white light, typically from a Mercury or Xenon arc lamp. Although such broad spectrum lamps can generate ample light at desired wavelengths, only a small percentage of the projected light is useful in any particular application. The other wavelengths need to be suppressed to avoid background noise that reduces image contrast and obscures the fluorescent light emissions.
This process of subtraction is complex, expensive and only partially effective: even after decades of refinements, the best filters are not 100% percent successful at blocking the bleed through of non-specific photons. Some mitigation techniques end up not only suppressing peripheral light, but also significantly diminishing the intensity of the desired wavelengths.
To address the root cause of the problem – the presence of non-specific photons – a radically different approach is coming to light.
Theoretically, a design that only introduces specific photons in the first place is preferable to a design that requires subsequent mitigation. In other words, an ideal light source would start with a baseline that contributes zero peripheral light and would allow the user to precisely control the addition of only those selective wavelengths of light that match the particular excitation wavelengths of the fluorochromes. The user should also be able to control the intensity and duration of the light in order to achieve the highest possible image quality while protecting the sample against bleaching and phototoxicity.
Recent advances in high performance Light Emitting Diode (LED) technology have enabled the practical implementation of this theoretical model. High-intensity monochromatic LEDs are now available in a variety of colors that match the excitation bandwidth of many commonly-used fluorescent dyes and proteins.

The Colibri LED light source system from Carl Zeiss MicroImaging employs a flexible modular design and up to four LED modules that can be used simultaneously in Colibri.
Carl Zeiss MicroImaging has incorporated this new LED technology in the Colibri illumination system, the new light source system for widefield fluorescence microscopy that uses specific wavelength windows with much less need to suppress unwanted peripheral wavelengths from a white light arc lamp. The modular Colibri system employs up to four LEDs, each individually and instantly controlled by electrical current without any of the mechanical switching devices like filterwheels or shutters required by traditional illumination systems. LEDs of different colors can be used in combination, giving users the option of seeing multiple fluorochromes simultaneously or rapidly capturing sequential images of each fluorochrome.

Ten different LED modules which can be easily exchanged are currently available for Colibri, from UV to dark red.
The Principle of Fluorescence
To understand how LEDs compare to other illumination technologies, it's useful to examine the illumination requirements of various fluorescence microscopy applications.
Fluorescence illumination is defined as the emission of photons by molecules whose electrons are transiently stimulated to a higher excitation state by radiation from an outside source. In other words, when a specific wavelength of light (the excitation wavelength) excites fluorescent dyes or proteins, the fluorescent material will appear to glow as it gives off light of a specific wavelength (the emission wavelength). The process is practically instantaneous: the emission occurs less than 10-6 seconds after the end of excitation.
Because energy is used in this process, the emission wavelength will always have less energy than the excitation wavelength, and by definition, lower energy light always has a higher wavelength. The difference in wavelengths between the excitation and emitted fluorescent photons is called the "Stokes Shift." Depending on the fluorochrome, the shift can range from just a few nanometers to several hundred nanometers.
Expanding Use of Fluorescence Microscopy
Fluorescence microscopy utilizes optical filters to separate excitation light from the emitted fluorescence, which is observed visually or detected by a camera equipped with a charge-coupled device (CCD) or other detectors. Fluorescence microscopy is an increasingly widespread medical and biological laboratory technique that provides highly sensitive detection for medical diagnostics and allows for the detection of cellular components and inter- and intra-cellular communication. Fluorescence microscopy is capable of detecting single molecules and sub-microscopic structures which are too small to be resolved by other conventional microscope techniques.
The availability of (green) fluorescent proteins (GFPs) derived from jellyfish, corals etc and its color-shifted genetic derivatives has greatly expanded the use of fluorescence microscopy during the past 10 years. Fluorescent proteins such as GFP are less phototoxic than the small fluorescent molecules in most chemical dyes such as fluorescein isothiocyanate (FITC), which can harm the specimen when illuminated during live cell fluorescence microscopy. This attribute of fluorescent proteins has inspired the development of highly-automated time-lapse live cell imaging systems.
Even more important is the fact that fluorescent PROTEINS can be expressed by the cells while other fluorescent dyes usually cannot penetrate the membranes of living cells. This limits their use mainly to fixed cells or needs microinjection, electroporation etc.
Light Source Requirements
The most basic requirement of a fluorescence microscopy light source is closely matching the excitation wavelength of the fluorochrome to achieve a high contrast image – that is, an image with a high signal-to-noise ratio. Wavelengths that match the fluorochrome strengthen the signal, but any peripheral wavelengths produce background noise that can overshadow the signal emitted by the object of interest.

Is this formula not a bit too simple? Other factors like detector noise, internal straylight etc contribute to the contrast as well.
A second and related requirement is illumination intensity, i.e., the number of photons specific to the excitation wavelength that reach the specimen. Obviously, the intensity must be sufficient to illuminate the objects of interest, but the optimal level of intensity will vary by application. The human eye is less sensitive than most automated detection systems, and therefore applications involving visual observation typically require higher levels of illumination intensity. On the other hand, lower intensity may be preferable in live cell imaging applications to protect against photobleaching and phototoxicity.
Intensity of illumination and signal intensity do not just follow a linear correlation. Saturation effects and dark states become more and more important with increasing illumination intensity.
An additional consideration is protection of the specimen, especially in live cell imaging applications. We have already mentioned the dangers of overexposure to light: phototoxicity of the specimen, and photobleaching of the fluorescent dye or protein. Overexposure can be avoided by attenuating the light intensity and by limiting the duration of the illumination to exactly the exposure time of the sensor. The cells also need to be protected from the heat generated by light source lamps and from the vibrations caused by mechanical filtering and switching devices.
Another factor to consider when evaluating light sources is the lifetime and stability of the lamp. Some light sources exhibit short-term intensity fluctuations and a substantial deterioration of performance over time. Limited lifespan results in more frequent bulb replacements, and poor stability diminishes the reproducibility of illumination conditions.
Traditional White Light Lamps
Mercury Lamps. Historically, Mercury-vapor high pressure arc lamps (commonly referred to as the HBO lamps) have been the most prevalent light source for fluorescence microscopy. An ecosystem grew around this illumination method during the past few decades, as chemical fluorescent dyes were developed and selected based upon conformance to the arbitrary (is ‘arbitrary' the right term?) emission peaks of the HBO spectrum, and many suitable filter sets became available.
Mercury light is characterized by very high emission peaks in the UV, blue and green regions, while in between the peaks the excitation energy of the light source is very low. The high excitation energy produces a bright signal from fluorochromes, making HBO a good solution for applications with weak staining (if the fluorochrome is stable enough to resist the effects of photobleaching). As mentioned above a weak staining will not result in a bright signal by just increasing the excitation intensity above a certain level. The high illumination intensity of Mercury lamps is difficult to attenuate, which increases the risk of phototoxicity of living cells.
The short life span of the HBO lamps (approximately 300 hours) necessitates relatively frequent bulb changes, which can be an expensive and cumbersome process. Newer systems simplify the adjustments required to align a new bulb or even automate this process.
Xenon Lamps. A second high-pressure white light source, Xenon-vapor arc lamps (XBO), produce relatively even emission levels across the visible spectrum. Absent the so-called Mercury peaks, the non-specific photons emitted by XBO are somewhat easier to suppress with filtration, and therefore XBO is often thought to provide better image contrast and to better protect living cells and tissue from phototoxicity.
While the flat output spectrum of the Xenon bulb means that it is significantly lower in intensity at the wavelengths typically associated with the Mercury peaks, the Mercury output can be up to 20 times brighter than the Xenon light source. However, outside of those peak areas, the intensity is comparable. One area of particular interest is the typical 480 to 500 nm excitation range of GFP, where Xenon has a similar intensity to that of mercury HBO.
The more even emission levels make XBO better suited to applications that compare the intensity between two different wavelengths, such as calcium experiments. Xenon lamps also have the advantage of more stable emissions over time. The lower fluctuations facilitate more reliable measurements in Ratio Imaging and other quantitative applications. The long time stability relates to the relatively long life span of XBO (400 to 2000 hours, depending on the bulb).
Fiber Coupled White Light Systems
Metal Halide. For many years, the decision about which light source to choose was limited: Mercury or Xenon. In the last five years, new types of light sources have been emerging in fluorescence microscopy. One of the first newcomers was the Metal Halide (HXP) white light source. HXP have a similar emission spectrum to Mercury, but in contrast to Mercury light sources, they are fiber-coupled to the microscope. The lamp and its power supply are housed in an external box, which is joined to the microscope, typically with a liquid light guide. The fiber-coupling reduces heat transfer from the light source to the microscope.
The HXP light sources provide high intensity comparable to HBO at Mercury peaks.
The liquid light guide acts as a scrambler to homogenize the arc output, eliminating the need to center the light and creating an even field of illumination without any need for alignment. HXP bulbs have a long lifetime, approaching 2000 hours, but the liquid light guide has a recommended lifetime of 18 to 24 months.
High Speed Xenon Systems. For live cell applications involving high speed image acquisition, several manufacturers offer external illumination systems utilizing a Xenon lamp with a fiber coupling to the microscope. These systems employ a combination of mechanical and optical techniques to achieve extremely high speed switching (1-3 milliseconds) to change intensity and wavelength. The fiber coupling has the advantage of reducing heat transfer from the light source to the microscope. The maximum illumination intensity is low and can be insufficient for visual observation.
Light-Emitting Diodes
A light-emitting diode is a compact semiconductor device that emits incoherent narrow-spectrum light when electrical current is applied. The color of the emitted light depends on the composition and condition of the semiconducting material used, and can be near-ultraviolet, visible, or infrared.
Unlike arc lamps that are inherently very bright, LED technology has dramatically evolved from humble beginnings. When the first commercial LEDs were introduced in 1968, they were capable of providing only 0.001 lumens of red light, a level of brightness suitable only for use as indicators. During the past four decades, LED technology has advanced at a rapid pace, comparable to the rate of advancement of microprocessors.
It is common knowledge that Gordon E. Moore predicted that the number of transistors on a chip would double about every two years. Less well known, except among LED engineers, is that a scientist at Agilent Technologies, Roland Haitz, predicted that the amount of light generated by an LED would increase by a factor of 20 every 10 years. "Haitz' Law" proved to be a reliable forecast, as LEDs have historically doubled in brightness every two years, and performance is predicted to continue to increase by 20 percent annually.
As LEDs have evolved in both brightness and in the range of available colors, they have been put to use in a wide variety of new applications. The most prevalent application is illumination as an energy-efficient and durable replacement for incandescent light bulbs. In fact, the U.S. Department of Energy has been investing heavily in this technology – $91.8 million in 2007 – as a core component of the federal government's energy conservation plans.
High performance LEDs are employed in many industrial, medical and military applications; for example, the Fiber Optic Gyroscope (FOG) is used in navigation and robotics and machine vision systems for automated manufacturing.
LEDs have been met with widespread acceptance in the field of medicine. High brightness LEDs are widely used for endoscopes and other invasive optical equipment. The highly specific light emissions make LEDs effective at diagnostic techniques like blood glucose measurement or pulse oxymetry, as well as for therapeutic applications such as acne therapy (blue or blue/red), neonatal jaundice therapy (blue), and photodynamic therapy when combined with specific drug treatment.
Advantages of LED in Fluorescence Microscopy
Now that high-performance LEDs provide sufficient intensity at the specific wavelengths required for many applications, fluorescence microscopy is able to take advantage of the benefits of LEDs, including their compact size, low power consumption, minimal heat output, fast switching and adjusting properties, high emission stability and extremely long life span.
The Colibri LED light source system from Carl Zeiss MicroImaging employs a flexible modular design and up to four LED modules that can be used simultaneously in Colibri. The beam paths from each module are steered into the microscope with a series of beam combiners, and 10 different LED modules are currently available for Colibri, from UV to dark red.

The modules can be easily exchanged by a user depending upon the experimental design of the day. The intensity of each module can be adjusted independently, precisely and reproducibly in percentage steps, so that for every fluorescent dye, the output emitted is precisely that which is needed to achieve the best possible compromise between the required excitation intensity and maximum sample protection. The modular design makes it possible to easily implement and exploit further developments in LED technology in the future.
An advantage of LEDs is that they instantly illuminate at full intensity as soon as electrical current is applied. Unlike arc lamps that are turned on continuously, LEDs can be switched on or off instantly when needed with no deleterious effects to their lifespan. Additionally, with no moving parts, the all-electronic system is vibration free.
Colibri is particularly well-suited for imaging applications requiring fast switching between wavelengths. Only some 300 microseconds are needed to switch between the LED modules.
The intensity of every LED module can be adjusted in percentage steps, enabling equidistant multichannel images to be easily realized in time-lapse-series. Instead of adapting the integration times of the camera to the illumination intensity, LED technology makes it possible to simply set the illumination intensity for the required integration time. The LED illumination intensity is also highly stable over time, making quantitative analyses easier and more reliable.
Summary
While the intensity of the LEDs has evolved significantly over the past few years, their intensity is still not as high as conventional arc lamps. However, in most live cell imaging environments, intensity from conventional light sources are typically reduced to minimize phototoxic affects to cells and tissues.
For applications requiring higher illumination intensity, or for applications requiring excitation wavelengths not currently supported by existing LED technology, Carl Zeiss MicroImaging offers a combination system that pairs a Colibri with an externally coupled HXP white light source. The Colibri control panel or the AxioVision software is used to switch over to and control the shutter of the HXP 120.
White light sources have been in use for decades, and much expertise has been developed in the ways of reducing the problems associated with peripheral light. Many labs have invested a great deal in filters used to suppress non-specific light. In the past, it may have been difficult to imagine that any other light source method would ever be viable.
Fortunately, a new type of light source is available. With LED technology, users can now take advantage of an excellent alternative for live cell imaging, high-speed or multi-channel fluorescence microscopy, and many other applications.
SOURCE: Carl Zeiss MicroImaging, Inc.